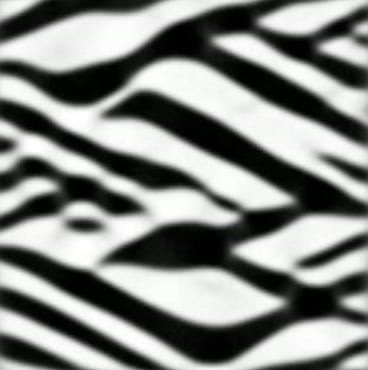
MIT team uncovers a reason why the hottest new material for rechargeable batteries works so well.
Since its discovery 15 years ago, lithium iron phosphate (LiFePO4) has become one of the most promising materials for rechargeable batteries because of its stability, durability, safety and ability to deliver a lot of power at once. It has been the focus of major research projects around the world, and a leading technology used in everything from power tools to electric vehicles. But despite this widespread interest, the reasons for lithium iron phosphate’s unusual charging and discharging characteristics have remained unclear.
Now, research by MIT associate professor of chemical engineering and mathematics Martin Z. Bazant has provided surprising new results showing that the material behaves quite differently than had been thought, helping to explain its performance and possibly opening the door to the discovery of even more effective battery materials.
When it was first discovered, lithium iron phosphate was considered useful only for low-power applications. Then, later developments — by researchers including MIT’s Yet-Ming Chiang, the Kyocera Professor of Ceramics — showed that its power capacity could be improved dramatically by using it in nanoparticle form, an approach that made it one of the best materials known for high-power applications.
But the reasons why nanoparticles of LiFePO4 worked so well remained elusive. It was widely believed that while being charged or discharged, the bulk material separated into different phases with very different concentrations of lithium; this phase separation, it was thought, limited the material’s power capacity. But the new research shows that, under many real-world conditions, this separation never happens.
Bazant’s theory predicts that above a critical current, the reaction is so fast that the material loses its tendency for the phase separation that happens at lower power levels. Just below the critical current, the material passes through a new “quasi-solid solution” state, where it “doesn’t have time to complete the phase separation,” he says. These characteristics help explain why this material is so good for rechargeable batteries, he says.
The findings resulted from a combination of theoretical analysis, computer modeling and laboratory experiments, Bazant explains — a cross-disciplinary approach that reflects his own joint appointments in MIT’s departments of chemical engineering and mathematics.
Previous analyses of this material had examined its behavior at a single point in time, ignoring the dynamics of its behavior. But Bazant and Cogswell studied how the material changes while in use, either while charging or discharging a battery — and its changing properties over time turned out to be crucial to understanding its performance.
“This hasn’t been done before,” Bazant says. What they found, he adds, is a whole new phenomenon, and one that could be important for understanding the performance of many battery materials — meaning this work could be significant even if lithium iron phosphate ends up being abandoned in favor of other new materials.
Researchers had thought that lithium gradually soaks into the particles from the outside in, producing a shrinking core of lithium-poor material at the center. What the MIT team found was quite different: At low current, the lithium forms straight parallel bands of enriched material within each particle, and the bands travel across the particles as they are charged up. But at higher electric-current levels, there is no separation at all, either in bands or in layers; instead, each particle soaks up the lithium all at once, transforming almost instantaneously from lithium-poor to lithium-rich.
The new finding helps explain lithium iron phosphate’s durability as well. When there are stripes of different phases present, the boundaries between those stripes are a source of strain that can cause cracking and a gradual degradation in performance. But when the whole material changes at once, there are no such boundaries and thus less degradation.
That’s an unusual finding, Bazant says: “Usually, if you’re doing something faster, you do more damage, but in this case it’s the opposite.” Similarly, he and Cogswell predict that operating at a slightly higher temperature would actually make the material last longer, which runs counter to typical material behavior.
In addition to seeing how the material changes over time, understanding how it works involved looking at the material at scales that others had not examined: While much analysis had been done at the level of atoms and molecules, it turned out that the key phenomena could only be seen at the scale of the nanoparticles themselves, Bazant says — many thousands of times larger. “It’s a size-dependent effect,” he says.
MIT materials science professor Gerbrand Ceder observed and wrote about lithium iron phosphate’s behavior at high current levels last year; now, Bazant’s theoretical analysis could lead to a broader understanding not only of this material, but also of others that may undergo similar changes.
Troy Farrell, an associate professor of mathematics at Queensland University of Technology in Australia, who was not involved in this work, says these findings are of great significance for those doing research on lithium batteries. He adds that this new understanding “enables material scientists to develop new structures and compounds that ultimately lead to batteries that have longer life and higher energy density. This is what is required if battery technology is to be used in high-power applications like electric vehicles.”
Understanding why lithium iron phosphate works so well was “one of the most interesting scientific puzzles I’ve encountered,” Bazant says. “It took five years to figure this out.”